As our world becomes increasingly interconnected and powered by technology, the role of GNSS technologies is becoming ever more important. From the coming wave of autonomous vehicles, to the revolution in surveying, to consumer level navigation, and more, GNSS is behind many of the everyday convivences you have come to appreciate. In this article we cover the differences between constellations, how your receiver calculates a position from a satellite signal and what the various signals do.
What enables this technology to work for us are GNSS receivers. A GNSS (Global Navigation Satellite System) receiver is an electronic device that is capable of receiving the signals broadcast by satellites to the Earth’s surface. These signals are used to calculate a precise and accurate position, or time that can be used in a variety of different applications.
- Understanding GNSS Basics
- GNSS Constellations and Signals
- GNSS Receiver Components
- Signal Acquisition and Processing
- Calculating a Position
- Common Error Sources
- GNSS Augmentation Systems
- Types of GNSS Receivers
- Where are we Headed with GNSS Technology?
1. Understanding GNSS Basics
In order to understand how a GNSS receiver works, we must first understand the basic concepts behind the concept of how these systems work. GNSS has revolutionized the way we navigate and determine our position on Earth. What these systems consist of is a constellation of satellites orbiting the Earth, with systems either covering the entire planet, or a localized region. Below, we will go over how they actually work
The Birth of GNSS
Although there are multiple different constellations in orbit today, GNSS began as the American GPS (Global Positioning System). Designed primarily for the military, it was originally designed to help determine the position of ballistic missile submarines during the 1960s. Once the potential of the system was seen, it was expanded until eventually in 1989 the United States released the Navstar system to civilian commercial airlines which has eventually involved into the GPS system we know now.
The Widespread Evolution and Adoption
If you are a country that considers itself a world player, you may not want to be beholden to the United States. As the United States is the sole controller of GPS, they also have the ability to withhold access to the system at any time. Obviously, this is not ideal. Therefore, in order to fulfill your ambitions, you will have to create your own GNSS system.
This line of thinking has led to the development of multiple other constellations, which fortunately is of great benefit to the rest of us, as it helps improve performance and accuracy. There are 3 other GNSS constellations, GLONASS, Galileo and BeiDou.
GLONASS is the Russian system, and is the oldest system of the of the three. Typically, older 3rd and 4th generation RTK systems are GPS and GLONASS only. Unlike the American system, the civilian signals on the GLONASS system are not upgraded as often. This means that unlike the other systems, GLONASS does not offer as high accuracy and “clean” signals.
Galileo is the system provided by the European Union. The EU system unlike the other systems discussed here is a civilian focused system, with signals designed to provide the best performance possible. With less of a military focus during the development, the Galileo system has several signal enhancements that are not available on other systems like the HAS (High Accuracy Service) observations that have been recently announced.
The final global system is the Chinese BeiDou system. At the time of writing the article, these are the best signals available to civilians. The recently launched Block III satellites provide the biggest difference between 6th and 7thgeneration RTK receivers. Depending where you are on the globe there are also several regional systems. This includes the Japanese QZSS system, which features incredibly high elevation satellites to help provide signals in deep urban canyons and the Indian IRNSS system, which is localized to the Indian sub-continent.
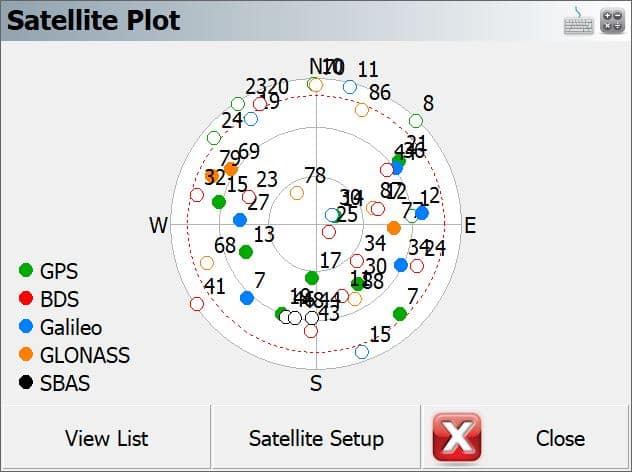
What Does a GNSS Satellite Do?
The last basic principle of GNSS is understanding what your GNSS receiver is actually receiving on the ground. Later in this article, we will go more into depth on how these signals actually work, and what factors can influence the signals before they reach your receiver.
A GNSS satellite works by continually broadcasting several pieces of information, including; orbital position, almanac information and timing information. This information is monitored by a number of ground stations to ensure that it remains accurate.
2. GNSS Constellations and Signals
In understanding what you can expect from a GNSS receiver, it is first important to understand in a little more depth each of the major constellations. We briefly covered them above, but we now get a little more in depth.
GPS (Global Positioning System)
- Number: 31 Operational Satellites
- Launch Date: 1978, Fully Operational in 1995
- Operator: United States Space Force
- Operating Altitude: 20,180 km
- Civilian Accuracy: 1 m / 3 ft 3 in (No DGPS or WAAS)
GPS is the GNSS constellation you probably think of first when thinking of navigation systems. It consists of worldwide coverage, and is available on nearly all civilian receivers.
GLONASS (Globalnaya Navigazionnaya Sputnikovaya Sistema)
- Number: 24 Operational Satellites
- Launch Date: 1982, fully operational 1995
- Operator: Russian Federation
- Operating Altitude: 19,130 km
- Civilian Accuracy: 2 – 4 m / 6 ft 7 in – 13 ft 1 in
The Russian equivalent developed in response to the GPS system. Used extensively in Russia and surrounding regions. One of the older systems, but has not been updated to the same extent as other constellations.
BeiDou (BDS)
- Number: 35 Operational Satellites
- Launch Date: 2000, fully operational 2018
- Operator: China National Space Administration
- Operating Altitude: 21,150 km
- Civilian Accuracy: 3.6 m / 12 ft
One of the latest constellation systems. Originated as a regional system over China, before being expanded to Global coverage. Some of the most accurate and powerful civilian signals available.
Galileo
- Number: 24 Operational Satellites + 6 More to Come
- Launch Date: 2005, fully operational 2016
- Operator: European Space Agency (ESA)
- Operating Altitude: 23,222 km
- Civilian Accuracy: 0.2 m or 7.9 in
Galileo is the European Union’s independent GNSS system designed for civilian and military use. It is known for a focus on precision and robust signal authentication features. Galileo is fully interoperable with GPS and GLONASS.
IRNSS (Indian Regional Navigation Satellite System)
- Number: 8 Operational Satellites
- Launch Date: 2013, fully operational 2018
- Operator: Indian Space Research Organisation
- Operating Altitude: 36,000 km
- Civilian Accuracy: 1 m or 3 ft 3 in
Primarily used for regional civilian applications in the Indian sub-continent, IRNSS is a geostationary constellation. What that means is that the satellites remain in a geostationary orbit providing coverage to the same region.
QZSS (Quasi-Zenith Satellite System)
- Number: 4 Operational Satellites
- Launch Date: 2018, fully operational 2018
- Operator: Japan Aerospace Exploration Agency
- Operating Altitude: 32,600 km and 39,000 km
- Civilian Accuracy: 1 m or 3 ft 3 in
QZSS is another regional satellite system designed to help enhance accuracy in the Asia Pacific region. The satellites are designed to help penetrate into urban canyons found in on the Japanese mainland that would be otherwise inaccessible to regular satellites.
GNSS Signals
In order to understand how a GNSS receiver actually works, we also have to understand what information it is actually using. There are multiple parts to the signals that are broadcast by a satellite.
GNSS Signals
The carrier frequency is the base radio frequency upon which GNSS signals are transmitted. It serves as the foundation for modulating data and navigation information onto the signal.
The carrier frequency is highly stable and precisely controlled, making it suitable for accurate time measurements. Different GNSS systems use distinct carrier frequencies, allowing receivers to identify which satellite signals they are receiving. Accurate measurement of the carrier phase is essential for high-precision positioning applications, such as Real-Time Kinematic (RTK) positioning.
Pseudorandom Noise (PRN) Codes
PRN codes are unique sequences of binary digits that are used to distinguish between different GNSS satellite signals. PRN codes are predictable and known in advance by GNSS receivers, enabling them to synchronize with the incoming signals. Each satellite broadcasts its own PRN code, allowing receivers to identify and track signals from multiple satellites simultaneously. PRN codes have specific lengths and periodicities, which are critical for signal processing and correlation.
Modulation
Modulation is the process of encoding navigation and timing data onto the carrier frequency. Modulation allows GNSS satellites to transmit essential navigation data, including satellite ephemeris (orbit information) and clock corrections. modulation schemes include Binary Phase Shift Keying (BPSK) and Quadrature Phase Shift Keying (QPSK), which are used to encode data on the carrier frequency. GNSS receivers use demodulation techniques to extract the navigation and timing information from the modulated signals.
Data Message
GNSS signals include data messages containing critical information about satellite orbits, clock corrections, and system status. Data messages are continuously updated and broadcast by the satellites to provide accurate and current information. Ephemeris data describes the precise orbits of each satellite, while almanac data provides approximate orbit information for all satellites in the constellation. The data message also includes information about the satellite’s health and the integrity of the transmitted signals.
Signal Structure
GNSS signals are structured with various components, including the carrier wave, PRN code, data message, and navigation bits. The structured signal allows GNSS receivers to synchronize with the incoming signal and precisely time the data extraction process. The navigation bits are transmitted at specific rates, and the structure ensures that the receiver can distinguish between data bits and other signal components.
Signal Bands
GNSS signals are transmitted on different frequency bands, such as L1, L2, and L5. Different frequency bands provide redundancy and mitigate ionospheric effects that can affect signal accuracy. Some GNSS receivers are capable of processing signals from multiple frequency bands to enhance accuracy and reliability.
Antenna Phase Center
GNSS antennas have phase centers, specific points on the antenna responsible for emitting and receiving signals. Accurate knowledge of the antenna phase center is crucial for antenna calibration and precise positioning.
3. GNSS Receiver Components
GNSS receivers are complex devices that require a lot of parts working in conjugation to generate a solution. The receiver must take the signals broadcast by GNSS satellites and convert them into electronic signals.
Antenna
The antenna is the first component that interacts with satellite signals. It receives signals transmitted by GNSS satellites and converts them into electrical signals. The antenna’s design is crucial for capturing signals effectively, and its performance can significantly impact the receiver’s accuracy.
Down-conversion
The down-conversion stage converts the received signals from their original frequencies (L-band for most GNSS systems) to intermediate frequencies (IF). This step simplifies signal processing and allows for better filtering and amplification of the signals.
Analog-to-Digital Converter (ADC)
The analog-to-digital converter digitizes the IF signals, converting them into digital data that can be processed by the receiver’s digital components. The bit depth and sampling rate of the ADC affect the receiver’s ability to capture and process weak signals accurately.
Digital Signal Processor (DSP)
The DSP is a critical component responsible for processing the digitized signals. It performs tasks such as signal tracking, demodulation, error correction, and the calculation of pseudo-ranges and carrier phases. The DSP extracts navigation data from the satellite signals and computes position and timing information.
Microcontroller or CPU
The microcontroller or central processing unit (CPU) manages the overall operation of the receiver. It handles tasks such as data storage, user interface, and communication with external devices. Advanced GNSS receivers may include a dedicated application processor for additional functionality.
Memory
GNSS receivers require memory for storing navigation data, almanacs, ephemerides, and user settings. Memory capacity is essential for efficient operation and providing rapid position fixes, especially in challenging signal environments.
User Interface
Many GNSS receivers include a user interface, which can be as simple as an LED display or as complex as a touchscreen interface. This interface allows users to interact with the receiver, view position information, view receiver status, and configure settings.
Communications Interface
GNSS receivers often have communication interfaces such as USB, Bluetooth, Wi-Fi, or serial ports to exchange data with external devices like computers, smartphones, or data loggers.
Power Supply
GNSS receivers require a power source, which can be provided by batteries, vehicle power supplies, or external power sources. Power efficiency is crucial for portable and battery-powered receivers.
External Sensors Optional
Some GNSS receivers may integrate external sensors such as accelerometers, gyroscopes, or magnetometers to enhance accuracy and provide additional data for applications like navigation and attitude determination.
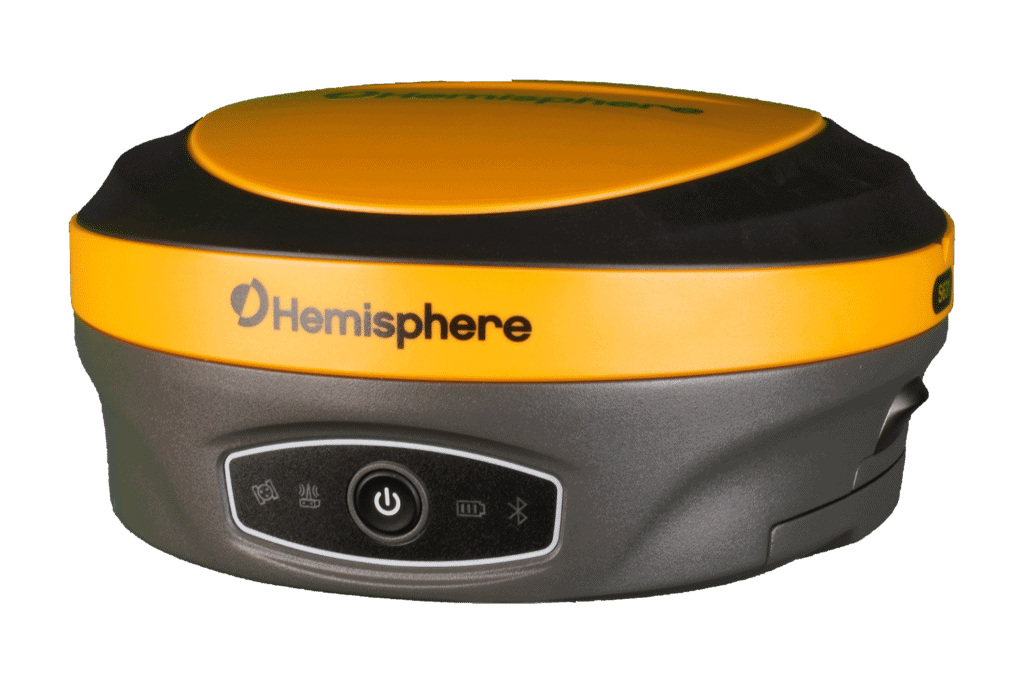
4. Signal Acquisition and Tracking
In order to make sense of a satellite signal, receivers must be not only capable of receiving satellite signals, they must also be able to process that information. The satellite signals must be acquired and tracked, and then decoded to extract the relevant information. There are several steps to this process.
Signal Acquisition
To begin, the receiver must search for satellite signals. Upon being turned on, the receiver immediately begins to search for signals. This is made easier by the fact that the receiver knows the approximate positions of the GNSS satellites in the sky, thanks to the satellite ephemerides and almanac data. This is why if older receiver has been turned off for a long time (think order of years), it may take up to a half hour for the receiver to reacquire the almanac data in order to calculate a position.
An RTK system can drastically increase the speed of a survey, especially when compared to a total station. As long as base corrections can be received, an accurate position can be established.
Once the receiver detects a satellite signal, it enters the tracking phase. During this phase, the receiver locks onto the signal and continuously tracks it as the satellite moves across the sky.
Signal Demodulation
The receiver demodulates the incoming signals, extracting the carrier frequency and pseudorandom noise (PRN) codes. These codes are unique to each satellite and are used for identification and timing.
Measurement Extraction
Pseudo-range Measurement: The receiver calculates the pseudo-range to each tracked satellite by measuring the time it takes for the signal to travel from the satellite to the receiver. This measurement is not yet precise because it includes errors. Think of it as the initial “guesstimate”.
Carrier Phase Measurement (Optional)
Some high-precision GNSS receivers also measure the carrier phase of the signal. Carrier phase measurements offer higher accuracy but require resolving the carrier cycle ambiguities. This is required in RTK measurements.
Error Corrections
There are a variety of error sources that can affect GNSS signals including atmospheric delays, clock errors, multipath reflections, and satellite orbit errors. All of these must be accounted for before a solution can be calculated.
In order to correct for these errors, the receiver applies error correction techniques to mitigate these errors and refine the pseudo-range measurements. On many high-end RTK receivers, this includes modelling the ionosphere to account for the atmospheric refraction on the signals.
Navigation Data Decoding
Each GNSS satellite transmits a navigation message that contains information about the satellite’s orbit, clock corrections, and other data. The receiver decodes the navigation message to obtain precise satellite position and timing information.
Position Calculation
To calculate the position, the receiver performs a trilateration calculation. The receiver does this by using the pseudo-range measurements to perform these trilateration calculations. By comparing the measured distances (pseudo-ranges) from at least four satellites with their known positions, the receiver can calculate its own three-dimensional position.
Velocity Calculation (Optional)
High-precision receivers can also use Doppler shift measurements to calculate velocity components along the line of sight to each satellite.
Time Calculation
The receiver’s clock is synchronized with GNSS time by accounting for clock biases and drift. Precise time information is essential for various applications, including synchronization and timing purposes.
Navigation Solution
The receiver combines the position, velocity, and time calculations to provide the user with a PVT solution, indicating where the receiver is located, how fast it’s moving, and what time it is, all with high accuracy.
Throughout the process, the receiver must contend with various challenges, including signal blockage (e.g., by buildings or natural obstacles), signal multipath (reflections), and atmospheric effects (e.g., ionospheric and tropospheric delays). Advanced GNSS receivers employ sophisticated algorithms and techniques to mitigate these challenges and provide accurate positioning and timing information.
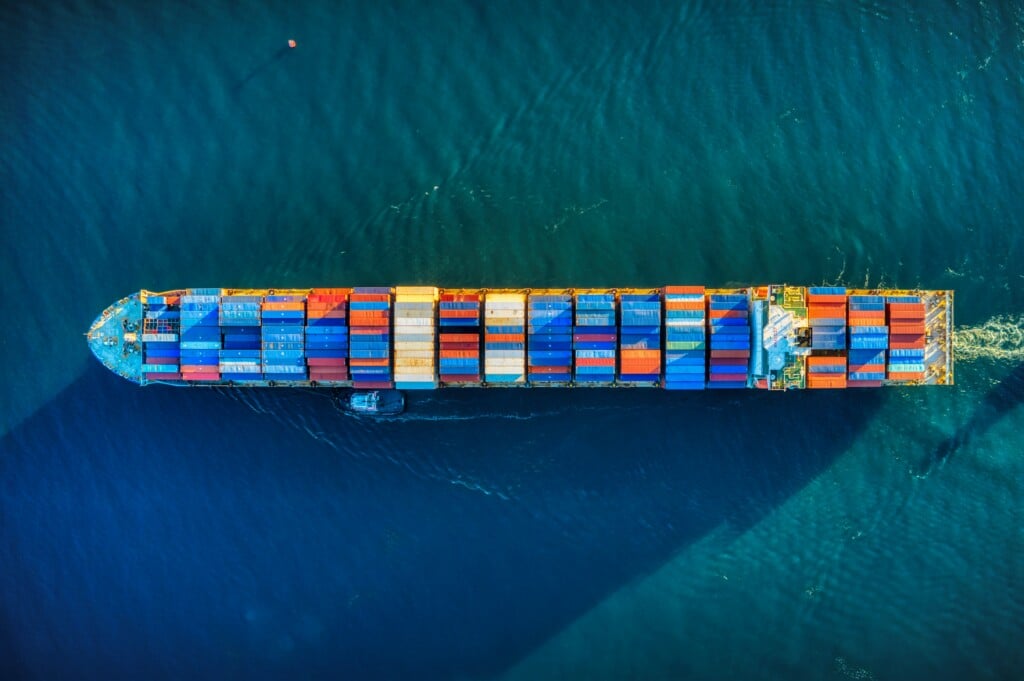
5. Calculating a Position
GNSS receivers rely on sophisticated algorithms that take millions of dollars to test and validate. These algorithms help determine a precise position on the Earth’s surface. The primary goal of these algorithms is to calculate the user’s position (latitude, longitude, and elevation).
Trilateration the Fundamental Algorithm
The fundamental positioning algorithm used in all GNSS receivers. Trilateration is a geometric method that determines a user’s position by measuring distances or pseudo-ranges to multiple known points in space (in our case the GNSS satellites). Here is how it works.
- Satellite Geometry
To perform trilateration, a GNSS receiver must receive signals from at least three satellites (four when altitude is required). Each satellite has an associated known position in space that it is broadcasting.
2. Pseudo-Range Measurement
The receiver then determines the time required for the signal to reach the receiver from the satellite. This time measurement is converted into a distance measurement, known as the pseudo-range. As an interesting aside, this technique was first put to the test during the First World War to locate enemy artillery using sound-ranging and famously employed at Vimy Ridge, part of the larger battle of Arras.
3. Possible Positions
Trilateration creates a sphere (3D) or circle (2D) around each satellite. The receiver is located somewhere on the surface of these spheres or circles because its distance to each satellite corresponds to the radius of the respective sphere or circle.
4. Intersection
The user’s position is determined by finding the intersection point(s) of these spheres or circles. In 2D, the intersection of three circles typically results in two possible solutions, and in 3D, the intersection of four spheres yields two possible solutions (the Earth’s surface and its antipode). Additional information, such as elevation or altitude, is used to select the correct solution.
5. Error Mitigation
Trilateration is subject to errors, including clock errors, atmospheric delays, and multipath reflections. To obtain accurate positions, advanced GNSS receivers use error correction techniques and data from additional satellites (more than the minimum required) for redundancy.
Advanced Position Techniques
While trilateration is the fundamental algorithm for GNSS positioning, advanced positioning techniques are employed to enhance accuracy and reliability:
- Differential GNSS (DGPS): DGPS uses a reference station with a known location to correct errors in the user’s position. This technique greatly improves accuracy.
- Real-Time Kinematic (RTK): RTK is a high-precision positioning technique that relies on a base station and rover. The base station transmits correction data to the rover, enabling centimeter-level accuracy.
- Carrier Phase Measurements: Advanced receivers use carrier phase measurements in addition to pseudo-ranges for higher precision. Carrier phase measurements provide sub-millimeter accuracy.
- Kalman Filtering: Kalman filtering is a mathematical technique used to estimate and predict a user’s position based on a combination of measurements, previous estimates, and known dynamics.
These advanced techniques, combined with trilateration, allow GNSS receivers to achieve high levels of accuracy and reliability, making them indispensable tools for various applications, from guiding vehicles to conducting precise surveys.
6. Common Error Sources
GNSS (Global Navigation Satellite System) measurements are subject to various sources of errors that can affect the accuracy and reliability of positioning and timing information. Here are some common sources of errors in GNSS measurements:
Atmospheric Effects
Ionospheric Delay: GNSS signals pass through the Earth’s ionosphere, which can cause signal delays due to the varying electron density. The delay is frequency-dependent and more pronounced at higher frequencies (e.g., L-band). Differential corrections can mitigate this error.
Tropospheric Delay: The troposphere also slows down GNSS signals due to factors like humidity, temperature, and pressure. Models and real-time weather data can help correct for this delay.
Multipath Reflections
Multipath occurs when GNSS signals bounce off surfaces such as buildings, water, or the ground before reaching the receiver’s antenna. These reflected signals can interfere with the direct signals, leading to measurement errors. Antenna design and signal processing techniques are used to mitigate multipath effects.
Buildings, trees, and terrain can obstruct GNSS signals, leading to signal blockage or signal weakening. In urban environments or dense forests, signal blockage is a significant challenge.
Receiver Clock Errors
GNSS receivers have onboard clocks that may not be perfectly synchronized with GNSS satellite clocks. Small clock errors can lead to timing inaccuracies, affecting positioning accuracy. Differential GNSS techniques help correct for clock errors.
Satellite Orbit Errors
Ephemeris Errors: The broadcast ephemeris data from satellites may contain errors in the predicted satellite orbits. These errors can lead to inaccuracies in position calculations. Precise ephemeris data from ground stations or real-time corrections can improve accuracy.
Satellite Geometry
The geometric arrangement of satellites in the sky can affect the accuracy of positioning. A poor satellite geometry, where satellites are clustered in one area of the sky, can result in dilution of precision (DOP) and less accurate position solutions.
Receiver Noise and Sensitivity
GNSS receivers are susceptible electronic noise, which can introduce errors in signal measurements. Higher-quality receivers with better sensitivity, signal processing, and shielding all of which can reduce the impact of noise.
Interference and Jamming
Interference from nearby electronic devices or sources can disrupt GNSS signals. Deliberate jamming or interference can also affect receiver performance. In fact, there was quite a stir when 5G was beginning to be introduced, as the frequencies were closely related to the existing GPS frequencies: Ligado 5G could be ‘harmful’ to older Pentagon satellite, report says.
To mitigate these errors and improve the accuracy of GNSS measurements, advanced techniques like differential GNSS (DGPS), real-time kinematic (RTK) positioning, and precise point positioning (PPP) are used. Additionally, the development of new GNSS constellations (e.g., Galileo, BeiDou) and modernization efforts aim to provide more accurate and reliable GNSS signals for users worldwide.
Error correction techniques, such as Differential GPS (DGPS) and Real-Time Kinematic (RTK) positioning, play a crucial role in improving the accuracy of GNSS measurements by mitigating common sources of errors. Here’s an explanation of these error correction techniques:
Differential GPS (DGPS)
DGPS is a technique used to correct GNSS measurement errors caused by factors such as atmospheric delays, satellite orbit errors, and clock discrepancies. It relies on the comparison of measurements made by a reference station with known coordinates to those made by a mobile or rover receiver.
A reference station, with precisely known coordinates, continuously receives signals from GNSS satellites. It calculates its own position based on these signals and calculates pseudo-range corrections for each satellite. The reference station broadcasts correction data, including pseudo-range corrections and, in some cases, carrier phase corrections, to nearby mobile receivers.
The mobile receiver, often referred to as the rover, receives the correction data from the reference station. It applies these corrections to its own pseudo-range measurements, reducing errors caused by factors like ionospheric and tropospheric delays.
By applying the correction data, the mobile receiver can significantly improve its position accuracy. DGPS can provide sub-meter to centimeter-level accuracy, making it suitable for applications like precision agriculture, surveying, and marine navigation.
DGPS requires access to a reference station with known coordinates, limiting its use to areas covered by such stations. It is also susceptible to errors if the reference station is too far from the mobile receiver.
Real-Time Kinematic (RTK) Positioning
RTK is an advanced GNSS positioning technique that offers extremely high accuracy, often within centimeters. It relies on a combination of rover and base stations, as well as carrier phase measurements.
A base station, with precisely known coordinates, continuously receives signals from GNSS satellites. It calculates its own position and the carrier phase corrections for each satellite. The rover receiver, often located on a moving vehicle or a surveyor’s tool, receives the carrier phase corrections from the base station in real time. These corrections are used to resolve carrier phase ambiguities.
Carrier phase measurements are more precise than pseudo-range measurements but are subject to integer ambiguities, which represent whole cycles of the carrier wave. RTK resolves these ambiguities using the corrections from the base station.
RTK can provide centimeter-level accuracy in real time, making it suitable for applications requiring high precision, such as land surveying, construction, and autonomous vehicles. RTK requires a nearby base station, which can limit its range of operation. Obstructions and signal blockage can also disrupt RTK measurements, so careful site planning is necessary.
Both DGPS and RTK are vital error correction techniques that enhance the accuracy and reliability of GNSS measurements in various applications. These techniques have revolutionized fields such as precision agriculture, land surveying, construction, and autonomous navigation, enabling precise positioning even in challenging environments.
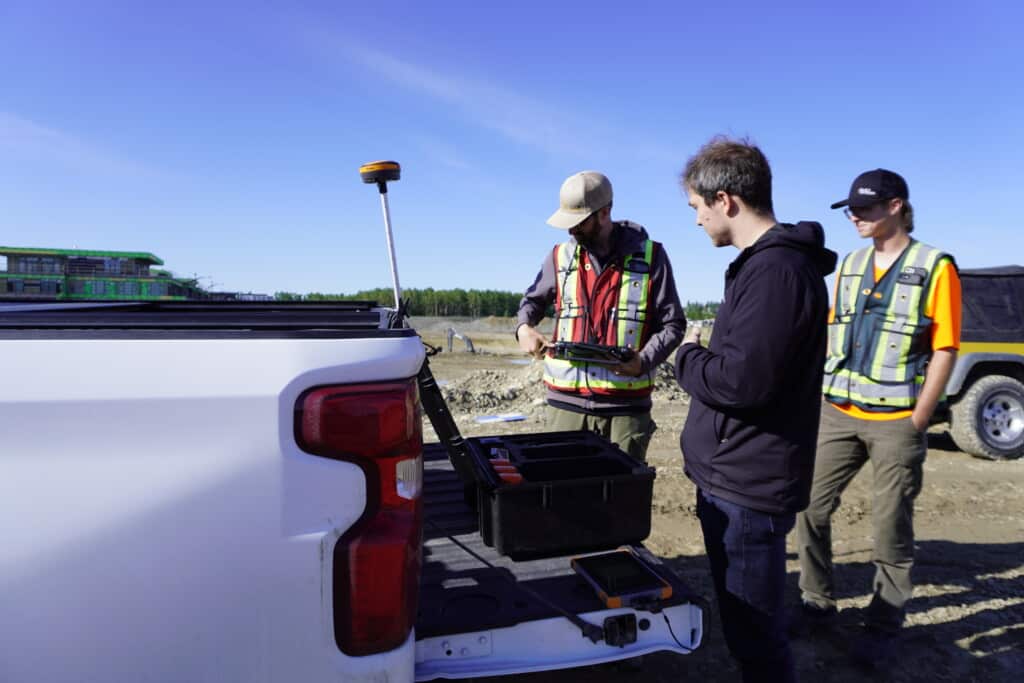
7. GNSS Augmentation Systems
In order to improve the reliability and accuracy of GNSS systems, many regional augemtation systems have been developed. They include systems like WAAS (Wide Area Augmentation System) and EGNOS (European Geostationary Navigation Overlay Service) and are designed to enhance the accuracy and reliability of GNSS (Global Navigation Satellite System) signals, particularly those from systems like GPS. Here’s an introduction to GNSS augmentation systems and how they augment accuracy and reliability:
Overview
GNSS augmentation systems are ground-based or space-based systems that work in conjunction with existing GNSS constellations (e.g., GPS, GLONASS, Galileo, BeiDou) to improve the accuracy, integrity, and availability of GNSS signals. These systems are especially important for applications that require high precision, such as aviation, maritime navigation, surveying, and agriculture.
Accuracy Enhancement: GNSS augmentation systems provide real-time corrections to GNSS signals, significantly improving position accuracy. This is crucial for applications where centimeter-level or sub-meter accuracy is required.
Integrity Monitoring: Augmentation systems continuously monitor the integrity of GNSS signals, detecting errors and anomalies. If a problem is detected, users are alerted, ensuring safety-critical applications have reliable information.
Increased Availability: Augmentation systems extend GNSS signal availability in regions where the signals from GNSS satellites may be weak or obstructed. This is achieved by broadcasting additional signals from ground-based or geostationary satellites.
Reliability: Augmentation systems enhance the reliability of GNSS positioning by reducing the impact of common sources of error, such as ionospheric and tropospheric delays, clock errors, and satellite orbit inaccuracies.
WAAS (Wide Area Augmentation System)
- Region: Primarily used in North America, but similar systems exist worldwide.
- Components: WAAS consists of ground-based reference stations, master stations, and geostationary satellites.
- How it Works: Reference stations monitor GNSS signals and send correction data to master stations. Master stations process the data and send corrections to geostationary satellites, which broadcast corrected signals to WAAS-enabled GNSS receivers.
- Accuracy: WAAS can improve the accuracy of GPS positioning to within 1-2 meters horizontally and vertically.
- Applications: Aviation, precision agriculture, surveying, and maritime navigation.
EGNOS (European Geostationary Navigation Overlay Service)
- Region: Primarily covers Europe.
- Components: EGNOS includes ground-based reference stations, a master control center, and geostationary satellites.
- How it Works: Similar to WAAS, reference stations monitor GNSS signals, and the master control center computes corrections. Geostationary satellites broadcast the corrections to EGNOS-compatible GNSS receivers.
- Accuracy: EGNOS improves the accuracy of GPS positioning to within 1-2 meters horizontally and vertically.
- Applications: Aviation, agriculture, surveying, road navigation, and search and rescue operations.
In summary, GNSS augmentation systems like WAAS and EGNOS play a critical role in enhancing the accuracy, reliability, and integrity of GNSS signals. They achieve this by continuously monitoring and correcting GNSS data, making these systems invaluable for applications that demand precision and safety, such as air and sea navigation, agriculture, and land surveying. These systems extend the usability of GNSS technology, even in challenging environments and regions with limited GNSS coverage.
8. Types of GNSS Receivers
1. Handheld GNSS Receivers
Handheld GNSS receivers are portable devices commonly used by hikers, outdoor enthusiasts, and geocachers and in GIS applications. They provide basic location information such as latitude, longitude, and altitude. And are generally accurate to 0.5 – 5 m. Receivers in this category include the Juniper Geode and Mesa 3 Geo.
2. Automotive GNSS Receivers
Automotive GNSS receivers are integrated into vehicles and provide real-time positioning information. They are commonly found in navigation systems and advanced driver assistance systems (ADAS). Another large and important industry is fleet management and tracking.
3. Aviation GNSS Receivers
Aviation GNSS receivers are highly specialized and designed to meet stringent safety and accuracy requirements for aircraft navigation. They often use multiple GNSS constellations for redundancy.
4. Maritime GNSS Receivers
Maritime GNSS receivers are used for navigation on water bodies. They are designed to withstand harsh marine environments and can provide additional data such as heading and speed. Hemisphere’s Vector line of receivers is an example of this.
5. Surveying and Geodetic GNSS Receivers
Surveying and geodetic GNSS receivers are highly precise instruments used in land surveying, construction, and geodesy. They can achieve centimeter-level accuracy, often in real-time. RTK systems, like the Hemisphere S631.
6. Agricultural GNSS Receivers
Agricultural GNSS receivers are used in precision agriculture to optimize farming operations. They help farmers with tasks like precise planting, harvesting, and soil analysis.
7. Military and Defense GNSS Receivers
Military-grade GNSS receivers are designed for secure and resilient positioning, navigation, and timing (PNT) in defense applications. They have anti-jamming and anti-spoofing capabilities and the ability to access encrypted signals unavailable to civilians.
9. Where Are we Headed with GNSS Technology?
1. Multi-Constellation and Multi-Frequency Support: GNSS receivers are increasingly capable of utilizing signals from multiple satellite constellations (e.g., GPS, Galileo, BeiDou) and multiple frequencies, improving accuracy and reliability.
2. High-Precision GNSS for Consumer Devices: Advancements are making high-precision GNSS technology more accessible to consumer devices, enhancing location-based services.
3. Real-Time Kinematic (RTK) and Precise Point Positioning (PPP): RTK and PPP techniques are becoming more common, offering centimeter-level accuracy for a wide range of applications.
4. Integration with Other Sensors: GNSS receivers are often integrated with inertial sensors, cameras, and LiDAR for improved positioning and navigation, especially in autonomous vehicles and drones.
5. Improved Urban and Indoor Positioning: Innovations are addressing the challenges of GNSS positioning in urban canyons and indoor environments, expanding its usability.
6. Cybersecurity: GNSS cybersecurity is a growing concern, leading to the development of secure and resilient GNSS receivers.
7. Miniaturization: GNSS receivers are getting smaller and more power-efficient, allowing integration into wearable devices, IoT sensors, and small-scale applications.
8. Enhanced Signal Processing: Advanced signal processing techniques are improving the accuracy of GNSS receivers in challenging environments.
These trends and innovations are driving the evolution of GNSS receiver technology, expanding its applications and capabilities across diverse industries while addressing the need for greater accuracy, reliability, and security.
Final Thoughts
GNSS receivers are the unsung heroes of modern navigation and positioning systems, leveraging signals from a constellation of satellites to provide us with precise location information. They operate on principles of triangulation, with advanced features like multi-constellation support and signal corrections from augmentation systems like WAAS and EGNOS, ensuring accuracy and reliability across various applications. GNSS technology has had a profound impact on our lives, from making every day navigation more convenient to revolutionizing industries like agriculture, transportation, and survey. As we look ahead, continued exploration and innovation in GNSS promise even higher precision, integration with emerging technologies, and novel applications, ensuring that GNSS remains a critical pillar of our interconnected world.
Let’s embrace these opportunities and contribute to the evolution of GNSS technology, shaping a future where accurate positioning and navigation empower industries, enhance safety, and drive scientific advancements while continuing to connect people and devices across the globe.
Bench Mark Equipment & Supplies is your team to trust with all your surveying equipment. We have been providing high-quality surveying equipment to land surveyors, engineers, construction, airborne and resource professionals since 2002. This helps establish ourselves as the go-to team in Calgary, Canada, and the USA. Plus, we provide a wide selection of equipment, including global navigation satellite systems, RTK GPS equipment, GNSS receivers, and more. We strive to provide the highest level of customer care and service for everyone. To speak to one of our team today, call us at 403-286-0333 or email us at [email protected].